
Research areas in the Snell Laboratory
The Snell Laboratory has been involved in a wide range of structural studies, may of which are summarized at the bottom of this page. However there are three main areas of interest, structural studies on static and dymanic targets, metalloproteins, and radiation chemistry and how it can be mitigated and used to study dynamic mechanism.
In a series of publications associated with the International Year of Crystallography, the Snell Laboratory discussed advancements in crystallization techniques and the construction of chemical approaches for obtaining crystals using pre-prepared crystallization screens. They also detailed the valuable information gained from visual outcomes of crystallization experiments and various methods for measuring these outcomes. The laboratory has studied crystallization across volume extremes, developing methods to optimize volumes for neutron diffraction studies and to minimize volumes for modern X-ray sources such as X-ray Free Electron Lasers. Proximity to the National Crystallization Center has provided numerous opportunities for extensive research in this field.
Uses of crystals have exploded and different techniques require different volume of crystals, from large for neutron studies, on the order of 10s of micros for synchrotron X-ray work, a few hundred nanometers for micro-electron diffraction, and down to the single molecule for small angle scattering or single particle cryo-electron microscopy. The laboratory works on understanding how to work with this entire range.
The Snell Laboratory has also been a pioneer in integrating machine learning and artificial intelligence technologies, creating one of the first comprehensive training sets for the automated classification of crystallization outcomes. This collaborative effort, involving research institutes, academia, government laboratories, the pharmaceutical industry, and GoogleBrain, resulted in a successful algorithm still used for classifying outcomes today. The potential predictive capabilities were highlighted in a review of computational crystallization approaches. Additionally, their investment in metadata capture during the Protein Structure Initiative led to early studies identifying physicochemical features of different crystallization mechanisms.
Crystallization screening involves diverse chemistries, often poorly captured in the literature and challenging to relate across multiple condition screens. In collaboration with the Collaborative Crystallization Centre in Australia and others, the Snell Laboratory helped establish the need for a consistent definition of crystallization components for effective machine learning. They demonstrated that identifying chemically similar and diverse conditions could be combined with outcome classification to pinpoint different optimization regions and mechanistic functions of targets.
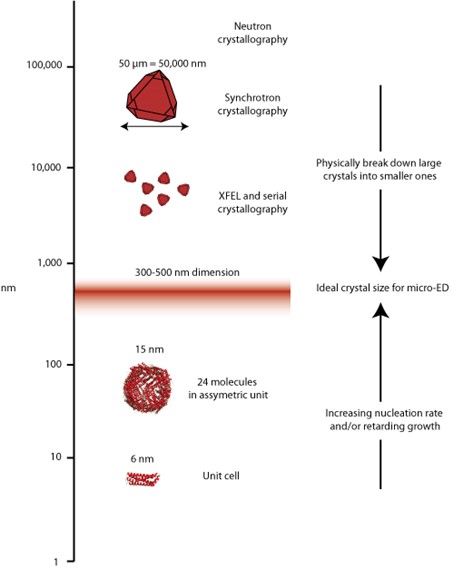
X-ray crystallography allows a well ordered protein to reveal its structural secrets. However, many proteins have areas withing them that are dynamic and flexible. Because of this the Snell Laboratory has made good use of Small Angle X-ray Scattering (SAXS) to image dynamics, develop procedures to ensure the quality of the date, and written the book on the subject.
The Snell Laboratory has also been a global leader in studying the impact of gravity on crystallization. Growing crystals in orbiting spacecraft reduces acceleration and minimizes convection over the crystal surface during growth. They developed sophisticated techniques to analyze the physical perfection of these space-grown crystals, discovering that while long-scale perfection (mosaicity) improves, short-scale perfection (atom-to-atom repeats) is strongly influenced by forces such as Brownian motion. This finding has implications for growing larger, more perfect crystals for neutron diffraction studies, although the benefit of a microgravity environment may be limited for improving short-scale quality with advancing X-ray sources.
Education is another area of significant interest for the laboratory. In an initiative designed to teach the scientific method to undergraduate students, various chemicals sourced from supermarkets were used as crystallization reagents with selected model proteins. Students developed hypotheses, made observations, and compared outcomes to existing literature. One notable case involved fish paste facilitating the crystallization of a target protein, which paralleled literature reports of crystallization using a specific amino acid. This experiment highlighted the protein’s selective crystallization contacts within the cocktail, providing a valuable teaching moment and a deeper understanding of the ‘silver-bullet’ approach to crystallization. Dr. Snell is actively involved in education, regularly teaching workshops around the world.
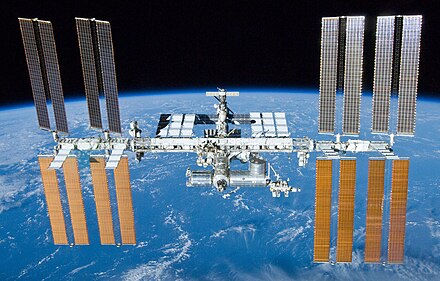
The Snell Laboratory has been analyzing the metal identity and content in metalloprotein samples used to obtain existing models in the Protein Data Bank (PDB). Techniques such as Particle Induced X-ray Emission (PIXE) and X-ray Fluorescence Spectroscopy (XRFS) at ion beams and synchrotron sources have been utilized. PIXE analysis of 87 metalloproteins revealed that 48% contained a metal in the model not present in the original protein sample. Of these, only 15% could be explained by promiscuous metals in the crystallization conditions, while 33% remained unexplained.
The laboratories X-ray fluorescence experiments at the Stanford Synchrotron Radiation Lightsource (SSRL), utilizing low X-ray absorption capillaries containing 400 nL of solution, with only 40 nL exposed to the beam. Remarkably, we successfully identified and measured metal content down to μM concentration. Leveraging 10 multiple exposures, we achieved a signal 8 times higher than noise for 1 μM Zn concentration. We further optimized automation for longer exposures and streamlined the process. Employing NIST trace standards for six individual metals and a mixture of four metals, we accurately determined metal identity and concentration down to 1 ppm for each sample. The NIST traceable single metal solutions, initially at a concentration of 1000 μg/ml, were appropriately diluted for the experiments (1x, 10x, 100x, and 1000x). We summed a total of 10 scans for 1x and 10x dilutions, 50 scans for 100x, and 100 scans for 1000x dilutions. This sensitivity of 1 ppm proves adequate for both identifying and quantifying the metal, comparable to PIXE capabilities. However, the requirement for 100x scans per individual sample proved time-consuming, while sample changeover was inconvenient. Additionally, data processing was time-intensive, and utilizing a regular crystallography beamline not tailored for such studies resulted in data of lower quality than could be achieved on a beamline dedicated to X-ray fluorescence. We have adopted an alternative approach that is just as sensitive and can evaluate hundreds of samples at a time. Work on this is in progress.
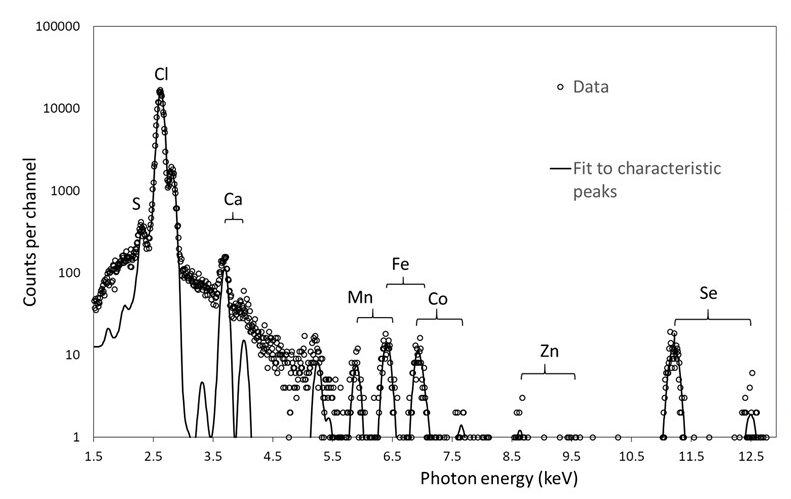
Spectrum showing PIXE data collected on a log scale and the calculated fit to the experimental data. Kα and Kβ emission peaks are seen for metals present. From Grime et al., 2020.
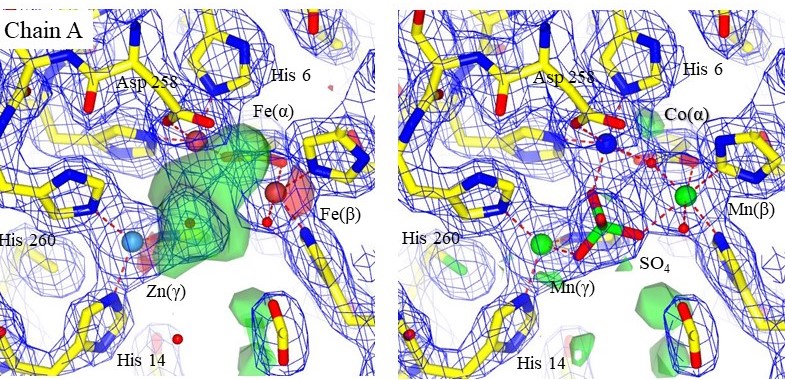
Structural models with electron density maps of the metal sites of one chain in 3DCP. The original metals are shown on the left, and the setals identified by PIXE on the right. An SO4 ion is clearly resolved in the electron density. Chains A and B were similar. Accurately modeling the correct metal can reveal mechanistic information that was otherwise missed. From Grime et al., 2020
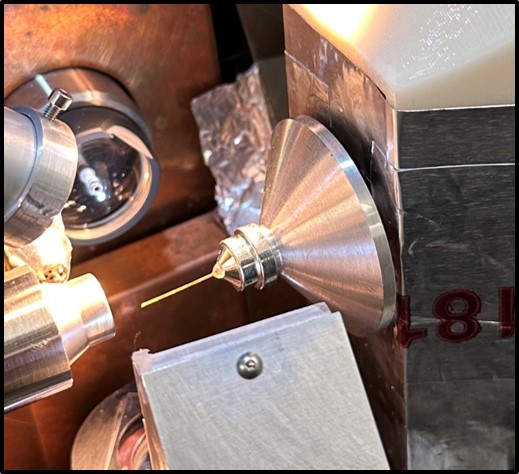
Low absorption X-ray tube in place at SSRL for X-ray flourescence measurements.
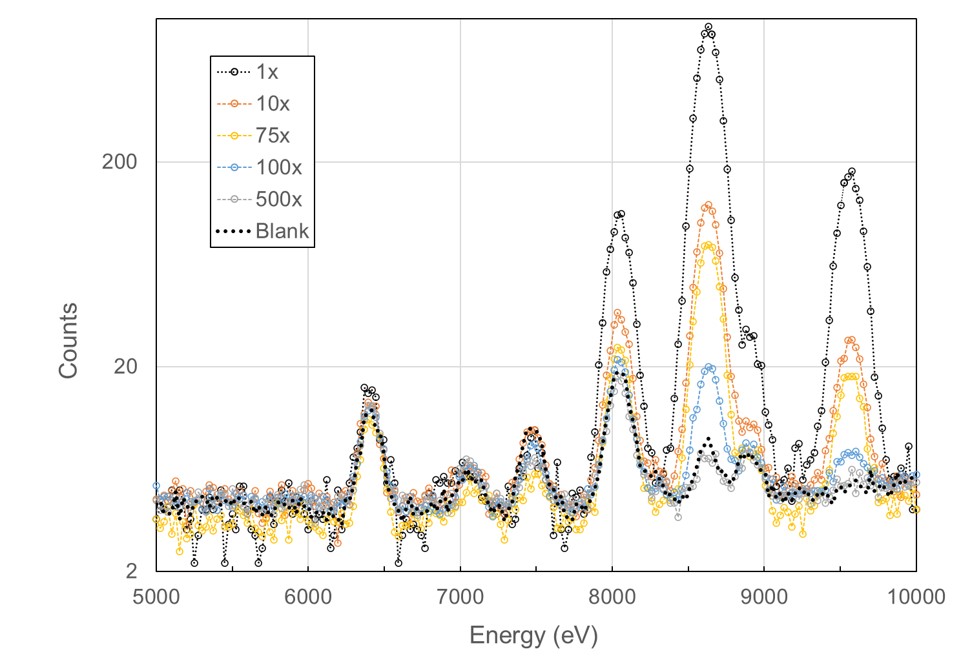
X-ray flourescence spectrum from 40 nL of a NIST standard trace metal water sample at different dilutions.
Incorrectly modeling a metal can lead to misunderstandings of mechanisms and functions. While many laboratories specializing in metalloprotein studies take great care to characterize the metals present, many laboratories producing metalloprotein structures are not specialists in this area. The problem is significant; in 2021, there were 1,465,753,416 models downloaded from the PDB, and conservatively, ~300 million of those were metalloproteins. PDB curators estimate that over 99% of downloads are not retrieved by structural biologists, and only ~4% are downloaded with their associated experimental data. Many researchers who use these models, both inside and outside the field of structural biology, may be unaware of these errors in metal identity or how to detect them. The growth of artificial intelligence and the use of these flawed data as training sets further exacerbate the problem, propagating inaccuracy. Metalloproteins are pivotal in disease progression and are promising targets for therapy. Misidentifying metals can lead to misconceptions about biological processes and hinder the development of treatments.
Radiation Chemistry and Biological Mechanism
Historically, the use of cryogenic temperatures in X-ray structural studies has been instrumental in mitigating damage to proteins caused by free radicals produced by the interaction of X-rays with the crystal. Additionally, it has enabled the trapping of intermediate steps in biological mechanisms, as seen in studies of superoxide dismutase. However, the cryocooling process introduces an additional step in the crystallography workflow, requiring the development of effective cryoprotectants. The Snell Laboratory has focused on methods to rapidly screen a large number of potential crystallization conditions and determine the appropriate cryoprotectant type and starting conditions. One such study, involving two summer interns, resulted in a paper with both interns as authors.
Questions about the cryocooling process itself—such as how cooling occurs and the impact of the X-ray beam’s energy on heating a cooled crystal—led the laboratory to use infrared imaging to observe the cooling process. The imaging revealed that the crystal cooled in a continuous wave from front to back when exposed to the cooling stream. When an intense X-ray beam hit the surrogate crystal, the same technique showed that heating was minimal, only a few degrees Kelvin at most.
The radiation chemistry process itself has both short- and long-range effects. Using electron paramagnetic resonance, UV spectroscopy, and X-ray crystallography, the laboratory developed a short-range model for the radiation chemistry associated with disulfide bond breakage, demonstrating both damage and repair processes. Data collected during this work, along with discussions about radiation chemistry impacts on other amino acids, helped develop a comprehensive model of damage in all amino acids.
Another target for this research was the enzyme xylose isomerase, which does not contain any disulfide bonds but has a bi-nuclear metal site. Previous research suggested that a metal shift was involved in the mechanism of action. However, the Snell Laboratory’s studies demonstrated that this apparent shift was simply a long-range radiation effect on the unit cell parameters, and the metals did not move. Neutron data, using a non-ionizing form of radiation, resolved the mechanism.
The National Academies’ 2022 consensus study report, “Leveraging Advances in Modern Science to Revitalize Low-Dose Radiation Research in the United States,” noted that “low dose and low dose-rate radiation effects on human health outcomes and the biological mechanisms of these effects are not fully understood.” One key finding was that “Radiation biology studies have contributed to the mechanistic understanding of the effects of radiation on molecular pathways and intra- and extracellular processes” and that “The application of novel and developing technologies will enable more precise definition of the cellular and molecular processes that are affected by low dose and low dose-rate exposures.”
The Snell Laboratory is particularly interested in low-dose radiation to mimic the biological processes involving free radicals naturally generated as part of biological function. They have explored biological systems that activate in response to radiation through damage to disulfide bonds and created proteins that can be used for low-dose studies, providing a structural response detectable by Small Angle X-ray Scattering (SAXS). This has enabled the development of strategies to minimize the biologically perceived dose while still obtaining sufficient signal-to-noise in the X-ray data to follow biological processes impacted by low-dose radiation and to study molecular processes in the body driven by free radical activation.
Other research areas
The research areas described above are very methodological. The methods developed and others employed have been used for biological based studies. These include studies of slidign clamp proteins, low resolution studies of integrin linked kinase, resolving the structure and function of eukaryotic glutaminyl-tRNA synthetase, enabling oligomeric protein interfaces to be distinguished with a hybrid SAXS and NMR approach, using terrahertz radiation to probe biological dynamics, studying the energy landscape of a cold unfolding protein with a battery of techniques, and contributing to our knowledge about SARS-CoV-2. All of the research has involved world wide collaborations.